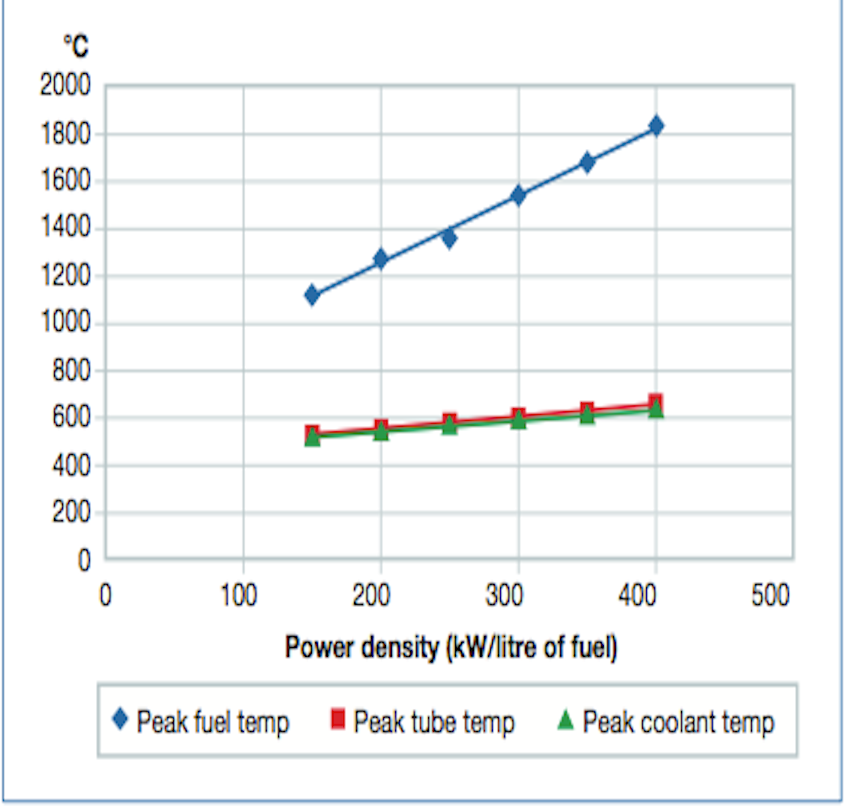
Molten salt nuclear fuel has a huge advantage over other fuel forms. The mixture of uranium or plutonium chlorides or fluorides with other halide salts causes the caesium and iodine fission products to form stable salts instead of remaining in the elemental form as happens in solid fuels. Elemental caesium and iodine are gases at reactor temperatures and it was the explosive release of these high pressure gases that made Chernobyl and Fukushima the continent wide disasters that they were instead of merely serious reactor accidents contained within their site boundaries.
As an alternative, but far safer, nuclear fuel, why are such fuels not just used instead of today’s solid fuels in fuel rods and fuel assemblies placed into a reactor core? Instead, until recently, every molten salt fuelled reactor concept used the same design paradigm where the fuel salt was also the coolant and has to be pumped out of the reactor core and through a heat exchanger. Designing and validating such systems, with their valves, emergency drain tanks, pumps, chemical treatment systems, fuel top up systems, degassing systems, etc, is a huge challenge in today’s regulatory environment where it must be proved to a high degree of certainty that systems will function as intended under all circumstances and for the life of the reactor.
The reason why the use of molten salt fuel in standard fuel assemblies – which greatly simplifies the design – has been neglected lies in a key decision made in 1949. The very first molten salt fuelled reactor, the Aircraft Reactor Experiment, looked at this possibility but rejected it. Being a plan for an aircraft engine, the designers knew they could not rely on convection as a heat transfer mechanism in the liquid fuel because the aircraft could experience free fall and convection would cease. Without a contribution from convection, heat would not pass out from the molten fuel fast enough to stop it boiling. A gravity independent pumped fuel system was adopted and this has dominated designers’ thinking ever since.
A contributory factor was that computational fluid dynamics (CFD) as a useable tool had yet to be developed so there was no way to calculate the contribution convection would have made. It was the application of this modern tool to the question that enabled the fundamental breakthrough on which the Stable Salt Reactor is based.
Thermal hydraulics of fission heat in fuel tubes
CFD simulations of heat transfer in metal tubes from fissioning molten salt fuel in the tube to a flowing molten salt coolant outside the tube were carried out using ANSYS/FLUENT by Wilde Analysis Ltd. The fuel salt simulated was a 60/40 mixture (mol%) of NaCl and UCl3. The thermophysical data for this mixture was taken from references 1 and 2. A typical vertical power distribution for a fast reactor core was used with a coolant flowing past the tube at 1 m/s. The coolant was 42%ZrF4/10%NaF/48%KF, with thermophysical properties taken from reference 3.
Simulations of tubes of different diameters showed that 10mm diameter tubes were optimal and those results are shown in Figure 1. Average power densities in the molten salt fuel as high as 300 kW/l could be achieved in a 10 mm diameter tube without reaching the fuel salt boiling point. With due conservatism (this is nuclear!) we selected a power density of just half this, 150 kW/l, allowing a comfortable margin of 500°C for power spikes.
Materials of construction and choice of salts
Molten salts have a reputation for being very corrosive to metals. The main reason for this is that they dissolve oxide films frommetals,anditisthoseoxidefilmsthat protect steels from corrosion by water or air. These “traditional” ways of creating corrosion resistant stainless steels are therefore not applicable.
Since there are no protecting films, the metal alloy itself must be chemically stable in terms of dissolution of its constituent metals into the salt. This dissolution is an oxidative process where the metal is converted to its halide salt. The main alloy component vulnerable to oxidation is chromium. Iron, nickel and molybdenum are all less easily oxidised. The tendency of a particular salt to dissolve chromium out of an alloy can readily be calculated based on the Gibbs Free Energy of the system. The key parameter to be measured is the equilibrium concentration of the chromium halide when the salt is in contact with an excess of chromium.
These calculations bring out a striking fact, which is illustrated in Figure 2. Uranium fluoride salts cannot be made sufficiently reducing (opposite of oxidising) to stop chromium dissolution without the uranium trifluoride undergoing disproportionation to uranium metal and uranium tetrafluoride. The limit of the ratio of UF3 to UF4 to avoid disproportionation is about 80/20 and as shown in the figure, chromium dissolves substantially into the salt under those conditions.
This does not mean that fluoride salts cannot be used, but their use will depend on developing special alloys. In nuclear, this represents a huge technical hurdle as years of “in reactor” testing would be required for any new alloy.
Uranium chloride salts do not however suffer from this problem. 100% UCl3 is stable with no tendency to disproportionate. But even 1% UCl4 renders it strongly corrosive – and fission causes a net release of chlorine to occur. The solution devised was to introduce metallic zirconium into the tubes containing the salt. Zirconium reacts avidly with UCl4 and ensures that the uranium salt remains very strongly reducing. The equilibrium concentration of CrCl2 when zirconium is present is less than 1 part per billion. Corrosion simply cannot happen.
This is hugely important in allowing rapid deployment of the Stable Salt Reactor. It means that existing alloys such as PE16, HT9 and other alloys developed and tested over the past 40 years for sodium fast reactors can be used in the Stable Salt Reactor.
Venting of fission gasses
Not all gaseous fission products form non volatile stable salts in molten salt fuel like iodine and caesium. The noble gases xenon and krypton are produced and there are hundreds of other possible volatile species. A key design decision was whether to trap these gases in the fuel tubes and allow them to build up substantial pressures or to vent them from the fuel tube into the reactor coolant.
Both options have history. The Dounreay Fast Reactor was a uranium metal fuelled, sodium cooled reactor and it allowed the fission gasses to be vented into the sodium. That was not a great success. Because it was a metal fuelled reactor, the caesium fission product was released as a gas from the fuel and contaminated the sodium coolant. It is only now, decades later, that that sodium has finally been disposed of. Later sodium fast reactors sealed their tubes and trapped the fission gases, which inevitably led to the tubes experiencing high pressures and the resulting risk of failure.
There seemed a possibility that the stable salt trapping capability of molten saltfuelmightcleanupthefissiongases enough to allow them to be vented. To investigate this, chemical thermodynamic calculations were carried out with the software package HSC8 to quantify the composition of the gas released during fission. The results are shown in Figure 3. At the high temperature of the fuel salt, about 1000°C, there are substantial amounts of gases released. But those gases immediately encounter the cooler walls of the tube above the level of the fuel salt where the temperature is the same as the coolant, 600°C. Since the gas flow is extremely slow, a few ml per day, there is plenty of time for the gases evolved to condense on the cooler walls. The result is that only Xe, Kr, Cd and ZrCl4 are released in significant quantities. None of these are hazardous radioisotopes – Xe-137, which decays to hazardous Cs-137, decays almost entirely (fraction remaining less than 10-6) while still in the tube gas space.
The decision was made to vent the tubes therefore as the hazard of pressurising the tubes was seen as greater than that of allowing the gases to vent into the inert gas containment of the reactor (not into the atmosphere). A recirculating gas cooler strips the condensable species (Cd, ZrCl4, coolant vapour) from the inert atmosphere to avoid them condensing on equipment within the gas containment, leaving only the noble gases.
Reactor design
Figure 4 shows an outline of the design for the reactor. It is a fast reactor with no moderator. This allows it to destroy higher actinides such as plutonium, americium and curium, which inevitably accumulate in thermal spectrum reactors. The design is, to the nuclear physicist’s eye, unusual. It is conventional to design fast reactors with small, very high power density, cylindrical cores. This is neutronically optimal in that it minimises the amount of fissile material required. Once upon a time, that choice made sense as fissile material was scarce and expensive. Today, plutonium in spent nuclear fuel (and purified plutonium such as that held at Sellafield in the UK) is a liability rather than an asset.
The neutronically suboptimal design shown has major compensating advantages. The rectangular core makes “on power” refuelling mechanically very simple. Fuel assemblies are migrated across the core with adjacent rows migrating in opposite directions. Fresh fuel assemblies are dropped in at one end of the row and spent assemblies removed from the other end.
The continuous refuelling capability allows the reactor to run virtually indefinitely without shut down, improving its economic efficiency and reducing the stress on components arising from thermal cycling. It also ensures that the reactor never contains excess fissile material that must be “damped down” with control rods or other reactivity controls. An entire high hazard failure mode is thereby eliminated.
The reactor core also operates at a medium power density, higher than a pressurised water reactor but lower than a sodium fast reactor. Again, this is neutronically somewhat inefficient but it makes cooling the reactor core far easier. Sodium fast reactors have to pump their sodium coolant at up to 10 m/s which results in high pressures, high stress on components, flow corrosion and increased risk of failures. The Stable Salt Reactor needs a coolant flow rate under 1 m/s with pumping pressures below 1 bar.
The coolant system consists of the primary coolant, as described above, which passes heat to an identical secondary coolant through heat exchangers immersed in the reactor tank. The primary coolant becomes radioactive due to neutron activation, the secondary coolant remains non radioactive and transfers heat out of the reactor building. A small positive pressure in the secondary coolant loop ensures no leakage of radioactivity out of the reactor in the event of a heat exchanger leak.
The reactor controls are exceptionally simple. Temperature and neutron sensors are provided around the core. Reactivity is controlled primarily by the refuelling system, with fresh fuel assemblies moved into the core only when reactor power has fallen enough to indicate the need. Emergency shut down is provided by essentially conventional control blades that are secured electromagnetically and drop into the core under gravity, passively in the event of power failure or actively in the event of the reactor exceeding its operatingparametersoradeliberateshut down.
Electricity generation system and GridReserve®
The reactor has an output temperature of ~600°C. The heat is transferred to the GridReserve® molten nitrate (solar) salt storage tanks, illustrated in Figure 5, which can hold 8 hours of continuous reactor output at 2.5 GW thermal. This is mature technology from the concentrated solar power industry [reference 4] and can be used to store energy at grid scale from any heat source that can provide the 550- 600°C temperature required. Conventional nuclear (300°C) cannot use this technology.
The nitrate salt is used to raise steam in boilers and standard superheated (575°C) steam turbines are used to generate electricity. This system allows a 2.5 GW thermal output reactor facility operating continuously to produce, for example, 2 GW of electricity for 8 hours a day, zero electricity for 8 hours and 1 GW for 8 hours, or select one of many alternative power production profiles. This takes the reactor out of the fast disappearing and relatively low value “baseload” market and allows it to provide a zero carbon complement to intermittent renewable energy sources. The economic value of this variable generationcapabilityisfargreaterthan the additional cost of the heat store, which adds just £3 per MWh to the levelised cost of electricity.
Reactor economics
Nuclear energy is becoming an “also ran” in the global power stakes. The International Energy Agency’s projection for electricity generating capacity worldwide by 2040 is for nuclear to account for only 350 GW outofatotalof10000GW–lessthan5%. That includes the ambitious nuclear plans of China. Meanwhile perfectly good, fully paid off nuclear power plants in the USA are being prematurely closed because they cannot compete economically with gas.
Nuclear can only make a real contribution if its cost falls dramatically – and the planet needs that to happen if greenhouse gas emissions are to be brought under control. Cost was therefore the primary driver in the design of the Stable Salt Reactor.
There are fundamental reasons why the Stable Salt Reactor should be radically cheaper to build than current reactors, which are:
- No high pressure nuclear systems. The requirement for extreme integrity standards for such systems makes them a major contributor to reactor costs.
- Smaller size. The Stable Salt Reactor with its containment is about one fifth the building volume per kW of the most compact PWR design in the world.
- Intrinsic safety as a consequence of fundamental science instead of multiple engineered controls and massive containment of major hazards.
- Simplicity of design and low component count. Not allowing nuclear physics optimisation to drive the design allowed for a radically simpler design – a highly cost effective trade off.
An estimate, carried out entirely independently of Moltex Energy by engineering company Atkins, gave a cost for the nuclear island of the reactor of just over £700 per kW. Extensions to this estimate by expert project civil engineers, with input from Siemens, for the non nuclear plant increased this estimate to £1300 per kW for the entire power plant (excluding GidReserve® system). That cost is less than one third that of a conventional reactor and suggests a levelised cost of electricity of under £30 per MWh, well below the current UK electricity wholesale price and less than one third of the UK Hinkley Point C strike price.
Next steps, and exciting competition
The Stable Salt Reactor is due to enter the Canadian Nuclear Safety Commission Vendor Design Review early in 2017 with a view to starting the licence process for a first of a kind commercial prototype in 2019 and operating by 2026.
There is an exciting competition developing between this concept for a molten salt fuelled reactor and the alternative use of “conventional” pumped molten salt fuel adopted by our friends at Terrestrial Energy. They have sought to address the engineering challenges of pumped molten salt reactors by replacing, decommissioning and disposing of their entire nuclear reactor system after just seven years of power generation. It is an interesting approach, with their nuclear system life similar to our fuel assembly life.
After half a century of no activity, this field of molten salt fuel has become truly dynamic.
References
- V N Desyatnik, S F Katyshev, S P Raspopin and Y F Chervinskii, Atomnaya Energiya, 39, 1975, pp 70-72
- G Long, UKAEA reactor group report AEEW R1059, 1970
- C Forsberg, American Nuclear Society annual meeting, June 2007
- Concentrated Solar Power Alliance technical report, Benefits of CSP with thermal storage, Sept 2014