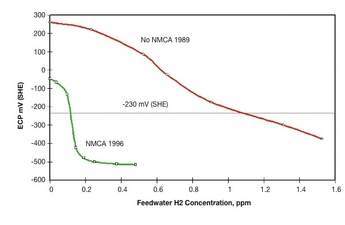
For more than three decades, operators of boiling water reactors have strived to mitigate intergranular stress corrosion cracking (IGSCC) in reactor internal components. IGSCC in the stainless steel piping, welds and reactor vessel internals of BWRs can reduce plant availability, increase inspection and repair costs, and has caused extended shutdowns at several BWRs. The cracking is caused by a combination of susceptible materials, an oxidizing environment, and welding and fabrication stresses. Greater understanding of the phenomenon, gained through fundamental research and operating experience, has enabled the Electric Power Research Institute, in collaboration with utilities and vendors, to develop and demonstrate chemical mitigation technologies to address IGSCC and protect susceptible BWR components.
These chemical mitigation technologies—hydrogen water chemistry (HWC), noble metal chemical application (NMCA), and Online NobleChem (OLNC)—reduce the oxidizing power of water by adding hydrogen to the feedwater, decreasing the electrochemical corrosion potential (ECP) of stainless steel surfaces and rendering them more resistant to IGSCC. An ECP value of less than -230 mV (vs. standard hydrogen electrode, or SHE) is required to achieve IGSCC mitigation.
More than 50 BWRs worldwide have monitored ECP under a variety of water chemistry conditions, and have taken control measures to achieve the desired ECP goal. However, ECP in the reactor environment varies depending on the water chemistry, radiation levels, and flow velocity at each location. It is of paramount importance to measure or estimate the ECP of the most conservative or controlling location to mitigate the risk of crack initiation, and of propagation of existing cracks, in all reactor internal materials. To achieve this objective, several BWRs have monitored ECPs in at least at eight different locations in the BWR heat transport circuit, which has enabled a better understanding of the oxidizing/reducing (redox) conditions prevailing in different parts of the reactor. The measurements have also supported improvements to ECP modelling efforts that can predict ECPs of locations that have not been well understood, such as the upper downcomer region of the BWR. With a judicious combination of ECP measurements and modelling, it has been possible to take adequate chemistry measures to maintain the ECP below the -230 mV(SHE) level at the controlling or the conservative location.
In 1991 EPRI published the Corrosion Potential (ECP) Measurement Sourcebook (NP-7142) summarizing the ECP information available at that time. During the past 20 years, major advances have been made in ECP monitoring technologies and methods that until now have not been documented nor made readily available to BWR operators. This month, EPRI will publish an updated version, BWRVIP-268: Electrochemical Corrosion Potential Sourcebook (EPRI Product 1025138). The new version will summarize the evolution of ECP monitoring developments from the early 1980s to the present, including a description of currently used and improved reference electrodes, monitoring approaches, and monitoring locations with representative ECP data from several selected plants that have employed chemical mitigation technologies such as HWC, NMCA, OLNC and non-hydrogen mitigation technologies.
Reference electrodes
ECP measurements are made by placing a reference electrode at the desired location in the BWR coolant stream and monitoring the potential difference between the reference electrode and any accessible point in the BWR vessel or piping using a high-input impedance voltmeter. The measured potential difference is converted to the SHE scale, using the theoretical potential of the reference electrode for comparison with measurements at other locations or with ECP data from other plants. Since the entire BWR vessel is grounded, the measured potential difference provides an indication of the ECP of the internal surface closest to the reference electrode location in that water chemistry environment.
ECP monitoring assemblies typically use two different types of reference electrodes: (1) iron/iron oxide (Fe/Fe3O4) and platinum (Pt), and (2) silver/silver chloride (Ag/AgCl) and platinum (Pt). The use of two types of reference electrodes allows ECP monitoring under both normal water chemistry (NWC) and hydrogen water chemistry (HWC) conditions and also enables checking one type of reference electrode against the other. The Ag/AgCl and Fe/Fe3O4 electrodes can be used for ECP measurements in both NWC and HWC over the entire range of feedwater hydrogen concentrations. Under NWC, there is no excess hydrogen in the BWR circuit, so a Pt electrode, which requires a stoichiometric excess amount of hydrogen, cannot be used as a reference electrode because its measurements of potential could be affected by the presence of oxidants like oxygen. However, under HWC conditions, where the Pt electrode behaves reversibly, it can be used as a reference electrode since the pH of the BWR water remains constant in the absence of chemistry transients.
Critical locations
The placement of ECP sensors in the BWR depends largely on which region is critical for SCC mitigation. The reference electrode should be placed as close as possible to the component that requires SCC mitigation to measure the corrosion potential of that location. Unfortunately, there are only several locations that are amenable for placing reference electrodes inside the BWR pressure vessel and the piping. These locations are summarized in order of preference below, and shown in Figure 1.

Figure 1: Schematic of typical BWR ECP monitoring locations
A. Lower plenum
ECP measurement at this location utilizes a fully-functional local power range monitor (LPRM) with three ECP sensors positioned well away from the active fuel zone. If a peripheral core LPRM location is utilized, the reference electrode can be placed to provide a representative ECP value for the bottom shroud welds (H8, H9, and H10). Similarly, the ECP of the bottom head shroud penetration welds also can be monitored, if the ECP sensors are placed inside the LPRM close to the bottom vessel welds.
B. Core support plate and top guide
This location utilizes a fully-functional instrumented LPRM with ECP sensors. The electrodes are aligned below or at the core plate elevation, immediately downstream of the cooling water holes in the LPRM cover tubes. Most BWRs have in-core monitor guide tube holes just below the core plate, and hence, this approach does not require machining of holes in the guide tube. The electrodes in this configuration operate in a significant radiation field from the core, and the current electrode designs are not capable of providing lifetimes exceeding 24 months.
C. Bottom head drain line flange
This location utilizes a three-inch stainless steel flange installed in the bottom head drain line (BHDL) or in a sample line off the BHDL to install ECP electrodes. In this case, water from the bottom plenum flows past the ECP sensors. The representativeness of the ECP data is directly related to the drain line flow and the transit time of the water from the bottom head to the BHDL flange location (to minimize decomposition of hydrogen peroxide).
D. Measurements of recirculation piping ECP in a decontamination flange
This location utilizes an existing decontamination flange to insert ECP sensors directly into the recirculation system. The electrodes remain recessed four to six inches inside the flange to protect them from any potential mechanical damage due to high flow impinging on the electrodes. The electrode configuration includes a support structure inside the flange that does not affect the flow conditions near the electrode tips. This location is excellent for measuring the ECP of the piping, but it does not provide ECP values representative of the in-vessel regions such as the core plate and the lower plenum.
E. Reactor water cleanup piping
This location uses an existing or newly-installed flange in the reactor water cleanup (RWCU) line in a location outside of the containment. The location has the advantage of providing more conservative ECP values than those in the recirculation piping, since it receives water from both recirculation piping and the lower plenum, but provides less conservative ECP values than those from the BHDL. The exact ECP measured depends on the ratio of the BHDL water to recirculation system water that contributes to the RWCU flow.
Using ECP to monitor water chemistry effectiveness
Continuous ECP monitoring has become important for HWC plants since ECP values can change depending on the timing of the operational fuel cycle. A typical example is the elevated ECPs observed in plants at the end of cycle when the core power peaks from bottom to the top, and the upper part of the core becomes more oxidizing. As a result, more hydrogen is required to achieve the same ECP at the end of cycle compared to the beginning of a fuel cycle (Figure 2). This information is crucial, and BWRs using continuous ECP monitoring have used this data to maintain feedwater hydrogen concentrations at the desired level to maintain ECP values below -230 mV(SHE)

Figure 2: End of cycle (EOC) ECP increase at constant feedwater hydrogen injection, as measured by two Pt electrodes at drain line ground location.
.
NMCA mitigates IGSCC at lower hydrogen injection rates and generates lower steam line radiation fields than moderate hydrogen water chemistry. The process involves the injection of small amounts of platinum and rhodium at a low temperature (235-300°F) followed by full-power operation with a small amount of hydrogen injection. The noble metal particles on internal surfaces catalyze the recombination of oxygen with hydrogen, thereby removing oxygen from the surfaces and decreasing the ECP at much lower feedwater hydrogen concentrations.
Figure 3 shows the lower core ECP data as a function of the feedwater hydrogen concentration at the Duane Arnold BWR with and without NMCA. The lower core ECP after NMCA decreased to almost -500 mV(SHE) at a feedwater hydrogen concentration of 0.2 ppm, while the 1989 data shows that the ECP before NMCA at the same hydrogen concentration was greater than +200 mV(SHE). The figure also illustrates that while 1.1 ppm feedwater hydrogen was required to achieve -230 mV(SHE) before NMCA treatment, < 0.15 ppm hydrogen is required to achieve the same ECP after NMCA treatment, with no increase in main steam line radiation levels in the long-term.

Figure 3: Lower core ECP response to HWC before and after NMCA at Duane Arnold
The OLNC process represents a further refinement of NMCA, involving the injection of small quantities of platinum into the feedwater piping during normal operation at full power. Whereas NMCA is typically applied every 4-6 years, OLNC is applied annually, and can be implemented after periods of off-hydrogen operation to treat the new crack surfaces with platinum and mitigate crack growth.
From the ECP data in Figure 4, low ECPs can be attained within 8 to 24 hours of platinum injection. The amount of platinum required to achieve low ECPs is in the range of 0.005 µg/cm2.

Figure 4: ECP Response of the recirculation flange and RWCU ECP monitoring location during OLNC.
Figure 5 shows the long-term ECP data at the recirculating piping surface location of Nine Mile Point 1 over several fuel cycles, where OLNC was applied at 11 to 16 month intervals. The ECP has remained below -380 mV(SHE) through several fuel cycles, showing the tenacity and durability of Pt-treated surfaces by the OLNC process.

Figure 5: ECP durability of OLNC-treated recirculating piping surface of Nine Mile Point 1, as measured by a Pt electrode at ground
Conclusion
The revised EPRI Sourcebook will provide BWR operators with a useful resource in designing and implementing ECP monitoring technology. Moreover, the Sourcebook will provide accurate interpretation and analysis of ECP data that will help plant operators mitigate IGSCC and maintain plant and component integrity under various chemistry regimes.
Author Info:
Susan Garcia, senior project manager, Electric Power Research Institute, 3420 Hillview Avenue, Palo Alto, California 94304
This article was first published in the December 2012 issue of Nuclear Engineering International magazine